HVAC SYSTEM SELECTION
- Scholenberg
- Nov 30, 2021
- 28 min read
Figure 1 shows a basic chilled water system with connected loads. The system consists of a chiller, cooling tower, building cooling load, chilled water and condensing water pumps and piping. This section will review each of the components.
1.0 Chiller Basics
The chiller can be water-cooled or air-cooled. The air-cooled chiller condensers can be either dry air or evaporatively cooled. The compressor types typically are reciprocating, scroll, screw, or centrifugal. The evaporator can be remote from the condensing section on air-cooled units. This has the advantage of allowing the chilled water loop to remain inside the building envelope when using an outdoor chiller. In applications where freezing conditions can be expected, keeping the chilled water loop inside the building avoids the need for some form of antifreeze. There can be multiple chillers in a chilled water plant. The details of various multiple chiller plant designs will be discussed in later sections.

The chilled water loop flows through the evaporator of the chiller. The evaporator is a heat exchanger where the chilled water transfers its sensible heat (the water temperature drops) to the refrigerant as latent energy (the refrigerant evaporates or boils). The refrigerant then gives up this heat to the condenser of the chiller. In the case of an air-cooled chiller, this heat is rejected to the outdoor environment via outdoor air being drawn through the condenser coils. For a water-cooled chiller, the refrigerant gives up its heat to a second water loop commonly referred to as the condenser water loop.
1.1 Flow and Capacity Calculations
For air conditioning applications, the common design conditions1 are 44°F supply water temperature and 54°F return water temperature resulting in a flow rate of 2.4 USgpm/ton. The temperature change in the fluid for either the condenser or the evaporator can be described using the following formula;
Q = W x C x ΔT
Where:
Q = quantity of heat exchanged, Btu/min
W = flow rate of fluid, lb/min
C = specific heat of fluid, Btu/lb•°F
ΔT = temperature change of fluid, °F
Assuming the fluid is water, the formula takes the form of:
Q = 500 x USgpm x ΔT for Q in Btu/hr
Q = (USgpm x ΔT)/24 for Q in Tons
Most air conditioning design conditions1 are based on 75°F and 50% relative humidity (RH) in the occupied space. The dew point for air at this condition is 55.1°F. Most HVAC designs are based on cooling the air to this dew point to maintain the proper RH in the space. Using a 10°F approach at the cooling coil means the supply of chilled water needs to be around 44°F or 45°F. The designer is not tied to these typical design conditions. In fact, more energy-efficient solutions can be found by modifying the design conditions, as the project requires. Changing the chilled water flow rate directly affects the chiller's performance. Too low a flow rate lowers the chiller efficiency and ultimately leads to laminar flow. The minimum flow rate is typically around 3 fps (feet per second) in the evaporator tubes. Too high a flow rate leads to vibration, noise, and tube erosion. The maximum flow rate is typically around 12 fps. The chilled water flow rate should be maintained between these limits of 3 to 12 fps. The condenser water flows through the condenser of the chiller. The condenser is also a heat exchanger. In this case, the heat absorbed from the building, plus the work from the compressor, transfers from the refrigerant (condensing the refrigerant) to the condenser water (raising the water temperature). The condenser has the same limitations to flow change as the evaporator.
1.2 Chillers and Energy Efficiency

Chiller part-load performance can be given at designer-specified conditions or the NPLV (NonStandard Part Load Value) can be used. The definition of NPLV is described in full detail in AHRI 550/590, Test Standard for Chillers
Since buildings rarely operate at design load conditions (typically less than 2% of the time) chiller part load performance is critical to good overall chiller plant performance. Chiller full and part load efficiencies have improved significantly over the last 10 years (Chillers with NPLVs of 0.35 kW/ton are available) to the point where future chiller plant energy performance will have to come from chiller plant design.
ASHRAE Standard 90.1includes mandatory efficiency requirements2 for minimum chiller performance. In recent versions of 90.1, different “paths” can be taken to comply with efficiencies as seen in Figure 2. Path A has efficiencies that represent a full load machine. This means that the majority of the hours that this chiller runs will be at full load and not at part load so the part-load efficiency does not need to be as low as Path B. Path B efficiencies allow the full load kW/ton to be higher because of the VFD on the compressor, however, the kW/ton for the part-load performance is much better because the chiller will be performing at part load the majority of the time.
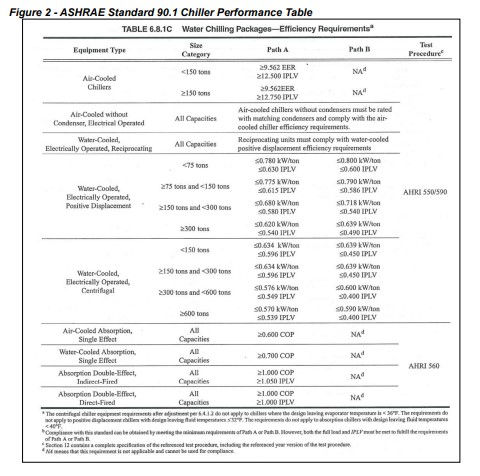
2.0 Piping Basics
The chilled water piping is usually a closed loop. A closed-loop only interacts with the atmosphere at the expansion tank. Figure 3 shows a simple closed-loop system. The static pressure created by the change in elevation is equal on both sides of the pump because the system is closed. In a closed-loop, the pump needs only to overcome the friction loss in the piping and through components. The pump does not need to “lift” the water to the top of the loop, just push the water through the system.

When open cooling towers are used in condenser piping, the loop is considered an open loop. The condenser pump must overcome the friction of the system and “lift” the water the elevation difference from the sump to the top of the cooling tower as shown in Figure 4. Note that the pump only needs to overcome the elevation difference of the cooling tower, not the entire building. If no isolating valves are used, the water in the system will seek a balancing point.
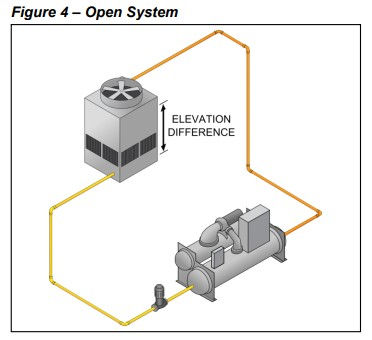
In the case of Figure 4, that balancing point is the bottom of the cooling tower sump. When the pump is shut off the water in the upper pumping will flow backwards to fill the sump. Special care should be taken for these situations, such as sizing the sump to hold the extra water in the system or isolating that side of the loop with a check valve. In high-rise applications, the static pressure can become considerable and exceed the pressure rating of the piping and the components such as chillers. Standard chillers typically handle 150 psi, but the reader is advised to check with the manufacturer. Although chillers can be built to higher pressure ratings, high-pressure systems can become very expensive. The next standard pressure rating is typically 300 psi. One solution

is to use heat exchangers to isolate the chillers from the static pressure. While this solves the pressure rating for the chiller, it introduces another hydronic device and another approach that affects supply water temperature and chiller performance. Another solution is to locate chiller plants on various floors throughout the building to avoid exceeding the 150 psi chiller pressure rating.
2.1 Expansion Tanks
An expansion tank is required in the chilled water loop to allow for the thermal expansion of the water. Expansion tanks can be an open type, closed type with the air-water interface or closed type with a diaphragm. The tank location will influence the type of expansion tank that should be used. Open tanks must be located above the highest point in the system (for example, the penthouse). Air-water interface and diaphragm type tanks can be located anywhere in the system. Generally, the lower the pressure in the tank, the smaller the tank needs to be.
The pressure at which the tank is operated is the reference point for the entire hydronic system. The location of the tank -which side on the pump (suction or discharge) - will affect the total pressure seen by the system as seen in Figure 5. When the pump is off, the tank will be exposed to the static

pressure plus the pressure due to thermal expansion. If the tank is located on the suction side, when the pump is running, the total pressure seen on the discharge side will be the pressure differential, created by the pump, added to the expansion tank pressure. If the expansion tank is located on the discharge side of the pump, the discharge pressure will be the same as the expansion tank pressure and the suction side pressure will be the expansion tank pressure minus the pump pressure differential.
2.2 Piping Insulation
Chilled water piping is insulated since the water and hence the piping is often below the dew point temperature. Condensate would form on it and heat loss would occur. The goal of the insulation is to minimize heat loss and maintain the outer surface above the ambient air dew point.
2.3 Condenser Water Piping
In most cases, the condenser water piping is an open loop. Figure 4 shows an open loop with the water open to the atmosphere. When the pump is not running, the level in the supply and return piping will be even at the level of the sump. When the pump operates, it needs to overcome the friction loss in the system and “lift” the water from the sump level to the top of the loop. Condenser water piping is typically not insulated since there will be negligible heat gain or loss and sweating will not occur due to the higher temperatures of the condenser loop. If the piping is exposed to cold ambient conditions, however, it could need to be insulated and heat traced to avoid freezing.
2.4 Reverse Return/Direct Return Piping

figure 6 shows reverse return piping. Reverse return piping is designed such that the path through any load is the same length and therefore has approximately the same fluid pressure drop. Reverse return piping is inherently self-balancing. It also requires more piping and consequently is more expensive. Direct return piping results in the load closest to the chiller plant having the shortest path and therefore the lowest fluid pressure drop. Depending on the piping design, the difference in pressure drops between a load near the chiller plant and a load at the end of the piping run can be substantial. Balancing valves will be required. The advantage of direct return piping is the cost savings of less piping. For proper control valve selection, it is necessary to know the pressure differential between the supply and return header (refer to Control Valve Basics). While at first, it would appear with reverse return piping, that the pressure drop would be the same for all devices, this is not certain. Changes in pipe sizing in the main headers, different lengths and fittings all lead to different pressure differentials for each device. When the device pressure drop is large relative to piping pressure losses, the difference is minimized.

In direct return piping, (Figure 7)the pressure drops for each device vary at design conditions depending on where they are in the system. The valve closest to the pumps will see nearly the entire pump head. Valves at the furthest end of the loop will see the minimum required pressure differential. Assuming the pressure differential sensor is located at the furthest end, all valves in a direct return system should be selected for the minimum pressure differential. This is because if anyone device is the only one operating, the pressure differential controller will maintain the minimum differential across that device. The decision of whether to use direct or reverse return piping should be based on system operability vs. first cost. Where direct return piping is used, flow-balancing valves should be carefully located so that the system can be balanced.
2.5 Piping and Energy Efficiency
Piping materials and design have a large influence on the system pressure drop, which in turn affects the pump work. Many of the decisions made in the piping system design will affect the operating cost of the chiller plant every hour the plant operates for the life of the building. When viewed from this life cycle point of view, any improvements that can lower the operating pressure drop should be considered. Some areas to consider are:
Pipe material. Different materials have different friction factors. Steel, PVC, and copper are typical piping materials for HVAC applications.
Pipe sizing. Smaller piping raises the pressure drop. This must be balanced against the capital cost and considered over the lifetime of the system.
Fittings. Minimize fittings as much as possible.
Valves. Valves represent large pressure drops and can be costly. Isolation and balancing valves should be strategically placed.
Direct return vs. Reverse return.
Piping insulation reduces heat gain into the chilled water. This has a compound effect. First, any cooling effect that is lost due to heat gain is an additional system load on the chiller plant. Second, in most cases, to account for the resultant temperature rise, the chilled water setpoint must be lowered to provide the correct supply water temperature at the coil. This increases the lift on the chillers and lowers their performance. ASHRAE Standard 90.1 dictates the specifics of hydronic systems in a building including specifics regarding minimum insulation that should be used on piping and how large of a pump is allowed in the building. The most recent version of Standard 90.1 should be referenced for the most up to date specific values. Table 1 shows the minimum piping insulation from Standard 90.13.

3.0 Pumping Basics

Typically centrifugal-type pumps, seen in Figure 8, are used for both condenser water and chilled water systems. They can be either inline or base mounted. The pumps must be sized to maintain the system dynamic head and the required flow rate. Normally, the pumps are located so they discharge into the chiller heat exchangers.

Centrifugal pumps do not function like positive displacement pumps; the centrifugal pump’s flow rate changes with the head. The actual operating point is where the system curve crosses the pump curve, as seen in Figure 9. In systems with control valves, the system curve changes every time a valve setting changes. This is important because the pump affinity laws cannot be used to estimate a change if the system curve is allowed to change. Identical pumps in parallel will double the flow at the same head. Identical pumps in series will double the head.
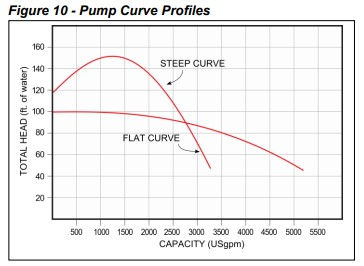
Figure 10 shows a steep and flat curve profile. Different pumps provide different profiles each with its own advantages. The steep curve is better suited for open systems such as cooling towers where high lift and stable flow are desirable. The flat profile is better suited for systems with control valves. The flat profile will maintain the necessary head over a wide flow range.

Figure 11 shows a typical pump curve. Red lines on the pump curve represent the different diameters of the impellers for the pumps. As the impeller increases, the amount of head that the pump can produce increases. The blue lines symbolize the system curve and how the system changes if a valve is opened or closed. For example, if a valve is closed, the dotted line to the left of the solid blue line might be used to represent an increase in the head requirements of the pump. The purple lines correspond to the brake horsepower of the pump. A pump with a specific impeller diameter running at a fixed speed will require a variation in horsepower as the head and flow change. While doing this, the efficiencies of the pump can also change. These are shown in green on the pump curve. While all of these parameters are being selected, there is also a requirement to keep enough pressure on the system to prevent cavitation (flashing the moving fluid into a gas), which damages the pump’s impeller. This requirement is called the Net Positive Suction Head (NPSH), shown in yellow on the pump curve. NPSH is an important consideration with condenser pumps particularly when the chillers are in the penthouse and the cooling towers are on the same level.
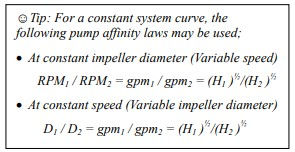
Since pumps are direct drive, the pump curves are typically for standard motor speeds (1200, 1800 or 3600 rpm). Once there is a selected pump speed, the required flow rate and head can be plotted on the pump curve. Where this point lays will determine what the impeller diameter, subsequent efficiency, and brake horsepower the pump will operate at.
3.1 Multiple Pumps
To provide redundancy, multiple pumps are used. Common approaches are (1) a complete full-sized stand-by pump, or (2) the design flow is met by two pumps with a third stand-by pump sized at half the load. When multiple pumps are used in parallel, check valves on the discharge of each pump are required to avoid “short-circuiting”. Pumps can also utilize common headers to allow one pump to serve multiple duties (headered primary pumps serving multiple chillers). Refer to the section on Primary Pumps for more information on primary pumps.
3.2 Variable Flow Pumps
Many applications require the flow to change in response to load. Modulating the flow can be accomplished by:
Riding the pump curve
Staging on pumps
Using variable frequency drives (VFDs)
Riding the pump curve is typically used on small systems with a limited flow range. Staging on pumps for small systems was the traditional method until VFDs. Staging pumps is a common method of control in large chiller plants where varying the tonnage in a plant is achieved by staging on chillers. Today, VFDs are the most common method for varying flow. They are the most efficient method as well. System flow is usually controlled by maintaining a pressure differential between the supply and return lines. The measuring point should be at or near the end of the pipe runs as opposed to being in the mechanical room to reduce unnecessary pump work. This is particularly true for direct return systems.

Figure 12 shows the differential pressure located at both the beginning and the end of the piping run. At design load, the pressure drop across coil 1 is 60 ft while the pressure drop across coil 5 is only 30 ft. The differential pressure controls, installed across the furthest coil in the system, should be set up to maintain 30 ft. When only coil 1 is operating, the pressure differential across coil 1 will only be 30 ft if the differential sensor is located at the end of the run as shown. If the sensors had been near the pumps, however, the differential controller would have to have been set for 60 ft to meet the design requirements. When only coil 1 operates, the pressure would have been maintained at 60 ft, which would have wasted pump work.
ASHRAE Standard 90.1 requires differential pressure setpoints to reset downward until at least one valve is 100% open. Initial setpoints for differential pressure may not be more than 110% of the design pressure needed for design flow through the critical load.
Another method of controlling variable flow pumps is to monitor the valve positions of a control valve in a critical part of the system. The control system then maintains the minimum pressure differential necessary, which allows the valve to maintain the setpoint. The advantage of this approach is the system pressure is maintained at the minimum required to operate properly and that translates into minimum pump work.
When multiple pumps are required to be variable flow, such as the secondary pumps of a primary-secondary system, VFDs are recommended on all pumps. If a system with two equal-sized parallel pumps does not have VFDs on both pumps, improper operation will occur when operating at part load. The VFD pump will slow down and generate less heat than the design conditions. The constant speed pump will then generate the design head and overpower the pump with the VFD.
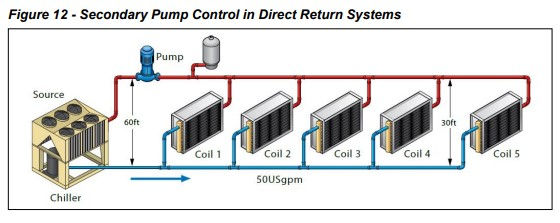
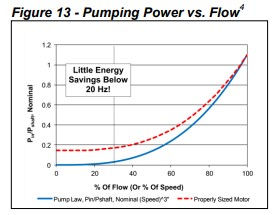
Figure 134 shows per cent pumping power as a function of per cent flow. From this figure, it can be seen that VFD pumps will not save much energy below 33% or 20Hz. Operating pumps much below 30% starts to create problems for motors, chiller minimum flows, etc. Since there are minimal savings anyway, the recommended minimum frequency is 20 Hz.
3.3 Pumps and Energy Efficiency
Pump work is deceptive. Although the motors tend to be small (when compared to chiller motors), they operate whenever the chiller operates. In a single water-cooled chiller plant with constant chilled water flow, it is not unusual for the pumps to use two-thirds of the energy consumed by the chiller. Optimal use of pumps can often save more energy than any other improvement to a chiller plant.
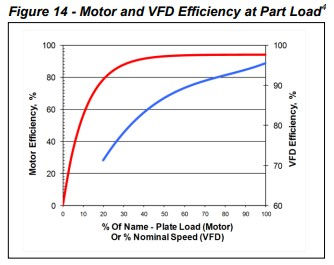
When both motors and VFDs operate at less than 100% capacity, their efficiency drops off. Figure 144 shows motor and VFD efficiencies at part load. It can be seen that oversizing motors can lead to significantly poorer performance than expected. Oversizing pumps themselves also lead to wasted energy. If the pumps produce too much flow, the flow will be throttled, usually with a balancing valve, to meet the desired flow. This creates an unnecessary pressure drop and consumes power all the time the pump operates. The solution in most cases is to trim the impeller. ASHRAE Standard 90.1 prescribes the operating conditions of pumps and VFDs. It specifies the maximum horsepower that is allowed in the building along with operating minimums for different sizes. Again, the most recent version of Standard 90.1 should be referenced for the most recent information.
4.0 Cooling Tower Basics
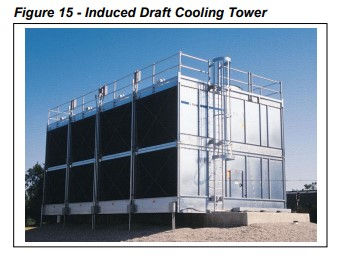
Cooling towers are used in conjunction with water-cooled chillers. Air-cooled chillers do not require cooling towers. A cooling tower rejects the heat collected from the building plus the work of compression from the chiller. There are two common forms used in the HVAC industry: induced draft (Figure 15) and forced draft (Figure 16). Induced draft towers have a large propeller fan at the top of the tower (discharge end) to draw air counterflow to the water. They require much smaller fan motors for the same capacity as forced draft towers. Induced draft towers are considered to be less susceptible to recirculation, which can result in reduced performance.
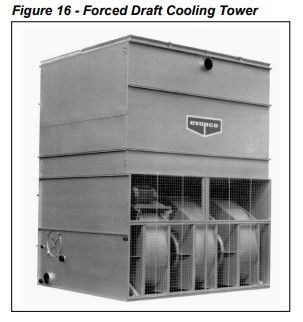
Forced draft towers have fans on the air inlet to push air either counterflow or crossflow to the movement of the water. Forward curved fans are often employed. They use more fan power than induced draft but can provide external static pressure when required. This can be important if the cooling tower requires ducting, discharge cap or another device that creates a pressure drop. Condenser water is dispersed through the tower through trays or nozzles. The water flows overfill within the tower, which greatly increases the air-to-water surface contact area. The water is collected into a sump, which can be integral to the tower or remote from the tower. The latter is popular in freezing climates where the condenser water can be stored indoors. Either tower type can have single or multiple cells. The cells can be headered together on both the supply and return side with isolation valves to separate the sections. This approach allows more cells to be added as more chillers are activated or to allow more tower surface area to be used by a single chiller to reduce fan work.
4.1 Typical Operating Conditions
The Cooling Tower Institute (CTI) rates cooling towers at 78°F ambient wet-bulb, 85°F supply water temperature and a 10°F range. Since it is common (but not necessary) to use a temperature range of 10°F, the cooling tower flow rate will be 3.0 GPM/ton compared to the chilled water flow rate which is 2.4 GPM/ton. The extra condenser water flow rate is required to accommodate the heat from the work of compression. Cooling towers are very versatile and can be used over a wide range of approaches, ranges, flows, and wet bulb temperatures. Lower condenser water temperatures can be produced in many climates with low wet-bulb temperatures which significantly improves chiller performance.
4.2 Cooling Tower Process
Cooling towers expose the condenser water directly to the ambient air in a process that resembles a waterfall. This process can cool condenser water to below ambient dry-bulb. The water is cooled by a combination of sensible and latent cooling. A portion of the water evaporates which provides latent cooling. The example below shows the cooling tower process on a psychrometric chart at AHRI conditions. As the wet-bulb temperature drops, cooling towers rely more on sensible cooling and less
on latent cooling. Ambient air below freezing can hold very little moisture which leads to large plumes; and in some cases, the winter tower selection requires a larger tower than the summer conditions. Additional care should be taken when selecting cooling towers for use in winter.
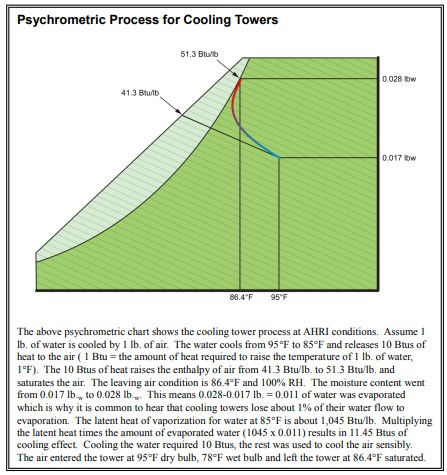
Approximately 1% of the design condenser water flow is evaporated (See the above example). A 1,000-ton chiller operating at design conditions can consume 1,800 gallons of water per hour. The specific amount can be calculated by reviewing the psychrometric process. In locations where the cost of water is an issue, air-cooled chillers may provide a better operating cost despite the lower chiller performance.
4.3 Winter Operation
Cooling towers that are required to work in freezing winter environments require additional care. The condenser water must not be allowed to freeze, particularly when the tower is idle. Common solutions include electric or steam injection heaters or a remote sump within the building envelope. The high RH of ambient winter air results in a plume, which can frost over surrounding surfaces. Low plume towers are available, but the freezing of condenser water on the tower itself can lead to blockage and reduced or no performance. Modulating water flow through a cooling tower (such as the use of three-way chiller head pressure control) should be given careful consideration. In many instances, this can lead to an increased possibility of freezing the tower.
4.4 Water Treatment
Condenser water has all the right ingredients for biological growth; it is warm, exposed to air, and provides a surface to grow on. In addition, the constant water loss makes water treatment even more difficult. Both chemical and ozone-based treatment systems are used. A thorough discussion on the topic of water treatment is beyond the scope of this application guide, but it suffices to say that it is necessary to provide the proper operation of both the tower and the chiller.
4.5 Closed Circuit Coolers
Cooling towers differ from closed-circuit coolers in that closed-circuit coolers reject heat sensibly while cooling towers reject heat latently. Consider ambient design conditions of 95°Fdb and 78°Fwb. If closed circuit coolers are used, the condenser water must be warmer than the ambient dry-bulb (typically 10°F warmer or 105°F). This raises the condensing pressure in the chiller and requires more overall power for cooling. Closed-circuit coolers are larger than cooling towers for the same capacity and can be difficult to locate on the roof.
4.6 Cooling Tower Controls
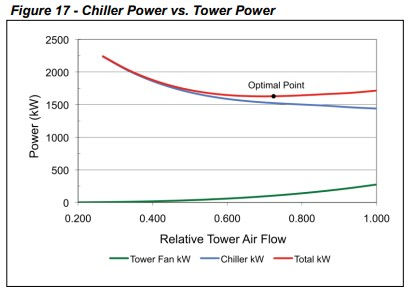
Cooling tower controls provide condenser water at the correct temperature to the chillers. Defining correct water temperature is very important. Lowering the condenser supply water temperature (water from the tower to the chiller) increases the effort by the cooling tower resulting in more fan work, however, this improves the performance of the chiller by offering condenser relief. Figure 17 shows the relationship between chiller and tower power. This chart shows how the power is affected by reduced tower airflow (higher ECWT) on the power requirement of an electric centrifugal chiller at full load.
Table 2 shows the range of chiller improvements that can be expected by lowering the condenser water supply temperature. The goal of cooling tower control is to find the balance point that provides the required cooling with the least use of power by the chiller plant.
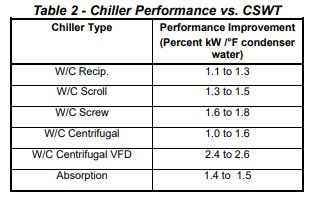
Cooling towers are often provided with aquastats. This is the most basic level of control. An aquastat is a type of temperature sensor that has a high-temperature setpoint and a low-temperature setpoint. If the water in the loop reaches a high temperature, it will trigger the condenser fan to come on until the water temperature reaches the low-temperature setpoint.
Aquastats are popular for single chiller–tower arrangements because the control package can be supplied as part of the cooling tower. The aquastat is installed in the supply (to the chiller) side of the cooling tower. In many cases, the setpoint is 85°F, which is very poor. If aquastats are going to be used, then a lower setpoint than 85°F should be used. One recommendation is to set the aquastat at the minimum condenser water temperature acceptable to the chiller. The cooling tower will then operate at maximum fan power and always provide the coldest possible (based on load and ambient wet bulb) condenser water to the chiller until the minimum setpoint is reached. Then the tower fan work will stage down and maintain minimum setpoint.
Figure 18 shows the 85°F setpoint and the AHRI condenser relief curve at which chillers are rated. Maintaining 85°F condenser water, while saving cooling tower fan work, will significantly penalize the chiller. There is some risk that without some condenser relief, the chiller may not operate at lower part-load conditions (chiller may surge). Minimum chiller setpoints are not a specific temperature. They change depending on the chiller load. A conservative number such as 65°F is recommended.
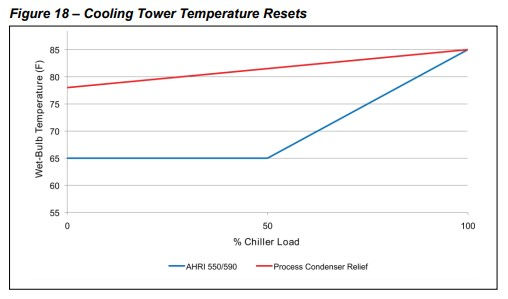
Another method to control cooling towers dedicated to single chillers is to use the chiller controller. Most chiller controllers today have standard outputs which can operate cooling towers, bypass valves and pumps. The chiller controller has the advantage of knowing just how much cooling is actually required by the chiller for optimum performance.
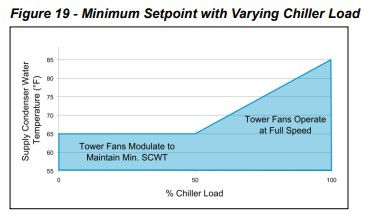
A method to control either single cell or multiple cell cooling towers serving multiple chillers is to base the condenser supply water temperature on ambient wet-bulb. For this method, set the condenser water setpoint at the current ambient wet-bulb plus the design approach temperature for the cooling tower. The set-point will change as the ambient wetbulb changes as seen in Figure 19. Limit the setpoint between the design condenser water temperature (typically 85°F) and the minimum condenser water temperature (typically 65°F).
The wet-bulb method will provide good condenser relief for the chiller and cooling tower fan work relief when the chiller is not operating at 100% capacity. This can be a good balance between chiller and tower work.
4.6 Head Pressure Control of Cooling Towers
Chillers that rely on oil for lubrication require a certain amount of pressure differential between the evaporator and the condenser to effectively “pump” the oil through the system to lubricate the bearings, gears, shaft seals, or create the seal between rotors and housing. Also, chillers that rely on refrigerants to cool the internal electrical components such as speed drives and motor windings need similar pressure differentials. All commercial water-cooled chillers operate on the premise that there will be higher pressure in the condenser than in the evaporator.
An effective condenser water temperature control system, or head pressure control, is the best way to ensure that the chiller will see enough pressure differential to start and operate correctly when there is very little heat in the evaporator water. Minimum condenser water temperatures for start-up vary by manufacturer and by type of technology, but all manufacturers of all types of technology recommend effective head pressure control.
The simplest and most common method of head pressure control is a water flow sensor in the condenser water. The chiller will not start unless this flow sensor proves that water is flowing through the condenser bundle. Some Daikin Applied chillers offer a feature called Lenient Flow which will allow the compressor to start for a short time before the flow sensor proves flow.
A more sophisticated control is a direct analogue output from the chiller controller which can operate a valve or a VFD to the condenser pump. The valve is generally a bypass type that bypasses condenser water around the cooling tower so it will heat up quickly and allow the compressor to start. The arrangements in Figure 20 are two examples of how this can be piped. Either method provides a constant water flow rate through the condenser tube bundle. The version located close to the tower also could be piped so that the bypassed condenser water goes directly to the tower cold water basin. This would provide some warmth to the basin water and would help prevent freezing in cold climates but would also require more time to heat up the condenser temperature to the appropriate level.

Another option for winter operation is to provide a sump within a heated building to accept the cold water from the tower, leaving the tower’s normal cold water basin empty.
The head pressure control could be a two-way valve in the condenser line which would directly vary the flow rate of condenser water entering the chiller by causing the condenser pump to “ride the pump curve”. The effect on the condenser system is to reduce the heat transfer both in the condenser bundle and the tower causing the refrigerant pressure to rise in the condenser. This approach has the same effect as using the chiller’s head pressure control signal to vary the speed of the condenser pump.
In all of these approaches, it is very important to establish a definite minimum flow through the condenser so that the water flow sensor will prove flow and allow the chiller to run. A default value for this minimum flow requirement is fifty per cent of the design condenser flow rate.
The chiller controller in most Daikin chillers includes an analogue output that is designed to directly control the VFD on cooling tower fans. It has the function of maintaining a specific condenser water temperature that leaves the cooling tower and enters the chiller condenser. The tower fan control signal must not be confused with the tower bypass control signal which prevents low-temperature condenser water from entering the condenser (initially set for 65°F). The tower fan control can control either the condenser entering water temperature ECWT or the pressure lift in the refrigeration cycle. Either control must be set up by a Daikin Factory Service Technician. Either staged ON/OFF fan starters (up to four fans) or a VFD can be operated by this controller.
Ultimately, the best cooling tower control designs are part of a chiller plant optimization program. These programs monitor the weather, the building load, and the power consumption of all the components in the chiller plant including cooling towers. Using modelling algorithms, the program calculates the best operating point to use the least power possible and meet the requirements of the building.
4.7 Cooling Towers and Energy Efficiency
Cooling towers consume power to operate the fans. Induced draft towers should be selected since they typically use half the fan horsepower force draft towers use. Some form of fan speed control is also recommended such as piggyback motors, multi-speed motors or Variable Speed Drives (VFDs). In addition, the sensor control logic is required to take advantage of the variable speeds.
ASHRAE Standard 90.1 necessitates that the cooling tower fan has to be able to be turned down and that the fan motor is limited to the specified horsepower. These values can be found in the most recent version of the standard. Depending on special situations, there are situations where the condenser fan does not need to have the capability to turn down. Again, these special situations should be reviewed in the most current version of the standard.
5.0 Load Basics
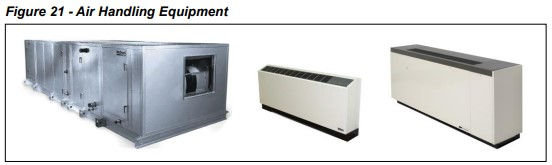
Chilled water coils are used to transfer the heat from the building air to the chilled water. The coils can be located in air handling units, fan coils, induction units, etc (see Figure 21). The air is cooled and dehumidified as it passes through the coils.
The chilled water temperature rises as the water takes heat out of the air that is passing over the coil. The cooling coil performance is not linear with the flow. Cooling coils perform 75% cooling with only 50% chilled water flow and 40% cooling with only 20% flow. As well, the leaving water temperature will approach the entering air temperature as the load is reduced.
Process loads can reject heat in the chilled water in a variety of ways. A common process load is a cooling jacket in machinery such as injection moulding equipment. Here the chilled water absorbs the sensible heat of the process.
6.0 Control Valve Basics
Control valves are used to maintain space temperature conditions by altering chilled water flow. Valves can be broken down into groups in several ways. Valves can be two-position or modulating. Two-position valves are either on or off and the control comes from time weighting. The percentage that the valve is open over a certain time period dictates the amount of cooling that the cooling coil actually does. Modulating valves vary the flow in response to a control signal such as a leaving air temperature.
Valves can also be classified as two-way or three-way types. Two-way valves throttle flow while three divert flow. Refer to the section on Piping Diversity for further explanation. There are several different physical types of valves. Globe valves, ball valves, and butterfly valves are all commonly used in the HVAC industry.
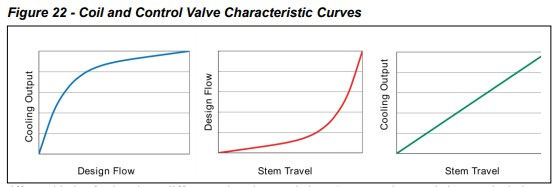
Different kinds of valves have different valve characteristics. Common characteristic types include linear, equal percentage, and quick opening. Control valves used with cooling coils need to have a performance characteristic that is “opposite” to the coil. Equal percentage control valves are typically used for two-way applications. For three-way applications, the equal percentage is used on the terminal port and linear is used on the bypass port.
Figure 22 shows an equal percentage control valve properly matched to a cooling coil. The result is that the valve stem movement is linear with the cooling coil capacity. In other words, a valve stroked 50% will provide 50% cooling.
6.1 Sizing Control Valves
Control valves must be sized correctly for the chilled water system to operate properly. An incorrectly sized control valve cannot only mean the device it serves will not operate properly; it can also lead to system-wide problems such as low delta T syndrome.
Control valves are typically sized based on the required valve coefficient, Cv. The Cv is the amount of 60°F water that will flow through the valve with a 1 psi pressure drop. The formula is:
G = Cv *ΔP ½
Where:
G = flow through the valve, USgpm
Cv = valve coefficient
ΔP = differential pressure required across the control valve, psi
The required flow at a control valve is defined by the needs of the device (fan coil, unit ventilator, or AHU) it serves. Cv values for valves are published by valve manufacturers. The required pressure differential through the valve is a difficult parameter to define. This is because the system can be either a reverse return piping system or a direct return piping system. The difference in the system selection will cause the pressure drops to be different at the same place in the system. While selecting control valves, attention should be given to this particular detail.

Figure 23 shows typical pressure drops from the supply to the return line for a cooling coil. For a modulating valve, the valve pressure drop should be as large of a percentage as possible when compared to the system pressure drop; preferably over 50%. The reason is to maintain valve authority. For on-off control, any valve can be used as long as it can pass the required flow rate with the pressure differential available.
6.2 Valve Authority
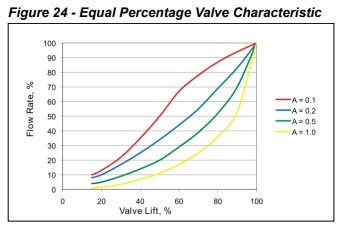
As a control valve closes, the pressure drop across the valve increases so that when the valve is completely closed, the differential pressure drop across the valve matches the pressure drop from the supply to the return line. This pressure drop is known as ΔPMax. When the valve is completely open, the pressure drop across the valve is at its lowest point and is referred to ΔPMin. The ratio of ΔPMin to ΔPMax is the valve authority, β. The increase in pressure drop across the valve as it closes is important to note. Valves are rated based on a constant pressure drop, as the pressure drop shifts, the performance of the valve changes. The method to minimize the change in valve performance is to maintain the valve authority (β) above 0.5.
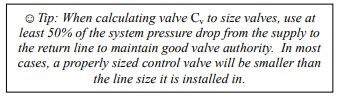
Figure 24 shows the change in the valve characteristic that occurs at different valve authorities. Since the goal is to provide a valve with a performance characteristic that is the opposite of a coil characteristic (See Figure 22), it is important to maintain the valve authority above 0.5.
6.3 Rangeablity
Rangeablity is a measure of the turndown a control valve can provide. The larger the range, the better the control at low loads. Typical ranges for control valves are 15:1 to 50:1.
6.4 Control Valve Location in Systems
Proper valve selection requires knowing the pressure drop from the supply to the return wherever the device is located. This information is typically not made available to the controls contractor which often leads to guessing. One solution would be for the designer to provide the required Cv for each valve. Another solution would be to provide the estimated pressure drops for each valve. Because the pressure drop from the supply to the return changes throughout the system, it can be expected that different valves, each with a different Cv, will be required. Even if all the coil flows and pressure drops were identical, the valves should change depending on the location in the system. Lack of attention to this detail can lead to low delta T syndrome (refer to Low Delta T Syndrome) that can be very difficult to resolve.

7.0 Loop Control Basics
There are two parameters that need to be considered for the chilled water loop. These are temperature and flow. The loop supply temperature is usually controlled at the chiller. The unit controller on the chiller will monitor and maintain the supply chilled water temperature (within its capacity range). The accuracy to which the chiller can maintain the setpoint is based on the chiller type, controller quality (a DDC controller with a PID loop is the best), compressor cycle times, the volume of fluid in the system, etc. Systems with fast-changing loads (especially process loads) and small fluid volumes (close-coupled) require special consideration.
The system flow control occurs at the load. To control the cooling effect at the load, two-way or three-way valves are used. Valve types are discussed in the section Control Valve Basics. Valve selection will also touch on piping diversity and variable vs. constant flow.
Another method to control cooling is face and bypass control at the air cooling coil while running chilled water through the coil. This approach has the advantage of improved dehumidification at part load and no waterside pressure drops due to control valves. The disadvantage is the requirement for continuous flow during any mechanical cooling load. In many cases, the pressure drop savings will offset the continuous operation penalty but only annual energy analysis will clarify it. Face and bypass coil control is popular with unit ventilator systems with their required high percentage of outdoor air and make-up air systems.
8.0 Piping Diversity
Diversity in piping is based on what type of valves are used. To maintain the correct space condition, three-way or two-way control valves are used. Three-way control valves (see Figure 25) direct chilled water either through or around the coil to maintain the desired condition. If all the loads on the loop use three-way valves, then the chilled water flow is constant. The temperature range varies directly with the load. That is if the design chilled water temperature range is 10°F, then every 10% drop in
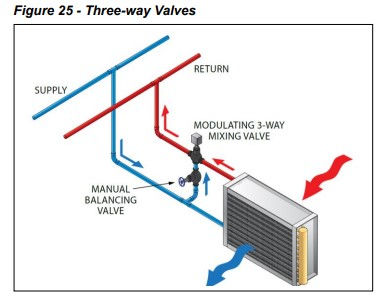
system load represents a 1°F drop in the temperature range. A system incorporating three-way control valves is easy to design and operate. The system pumps all the water all the time, however, this requires more pump horsepower. In most cases, the chiller is sized for the building’s coincident load or peak load. Due to diversity, not all the connected loads will “peak” at the same time as the building peak load. However, the pumps and piping system must be designed for full flow to all the control valves all the time. Since the chiller flow rate is the same as the flow rate through all the loads (they’re connected by the same piping system and pump) the diversity is applied to the chiller temperature range.
For example, consider a building with an 80-ton peak load. Summing all the connected loads adds up to 100 tons. In short, this building has a diversity of 80%. Using a temperature range of 10°F at each control valve, the total system flow rate is:
Flow = 24 x 100 tons/10°F = 240 GPM
However, an 80-ton chiller with 240 GPM will only have a temperature range of 8°F. The lower chiller temperature range is not a problem for the chiller operation, but it will lower the chiller efficiency.
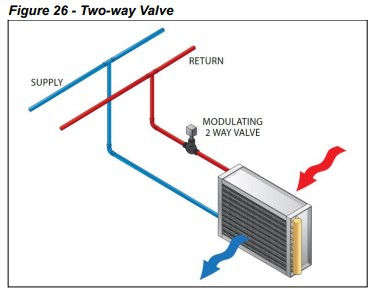
Care must be taken to select the chiller at the proper temperature range.
When two-way modulating control valves (see Figure 26) are used, the flow to the coil is -restricted rather than bypassed. If all the valves in the system are two-way type, the flow will vary with the load. If the valves are properly selected, the temperature range remains constant and the flow varies directly with the load. In this case, the diversity is applied to the chilled water flow rate.
Using the previous example, the peak load is 80 tons and the design flow is 2.4 x 80 tons or 192 GPM. The connected load is still 100 tons and requires 240 GPM if all the two-way control valves are open at the same time. The 80% diversity assumes only 80% of the valves will be open at the peak load.
The advantage of two-way control valves is both the pump and the piping are sized for a smaller flow rate, offering both first cost and operating savings. The difficulty is that the chiller and control system must be designed for variable flow. The chiller has a minimum flow rate so the piping design has to a05llow for enough flow during all operating conditions to meet the chiller minimum flow rate. Using two-way valves is the main building block for a variable flow system
HVAC Repair Diamond Bar company's commitment to customer satisfaction was evident in their diligent approach to system selection. They patiently answered our questions and provided valuable insights, making the decision-making process smooth and stress-free.